Environmental Impact of Thermodynamic Processes: Analysis for Sustainable Engineering Solutions
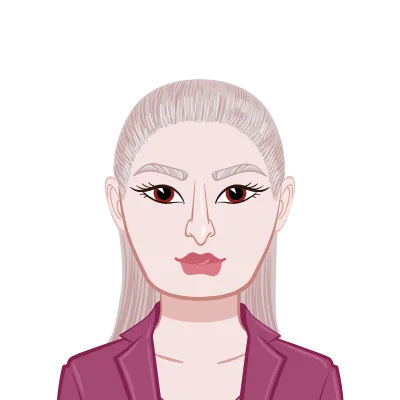
Thermodynamic processes stand as the bedrock of mechanical engineering, defining the principles by which energy is harnessed, transformed, and utilized to power an array of systems integral to our daily lives. At the core of mechanical engineering, these processes govern the operation of engines, refrigeration systems, and other mechanical systems that propel innovation and progress. Whether fueling the engines of transportation or generating electricity through turbines, the understanding and optimization of thermodynamic processes are fundamental to the design and functionality of an extensive range of mechanical systems.
Amid the undeniable prowess of thermodynamics in driving technological advancement, an escalating concern is carving out a prominent niche within the engineering landscape—the imperative for environmental sustainability. The traditional tenets of mechanical engineering, while delivering remarkable efficiency and performance, often exact an environmental toll. As our planet grapples with the far-reaching consequences of climate change and environmental degradation, the engineering community is facing a paradigm shift. No longer can the efficacy of mechanical systems be evaluated in isolation from their environmental repercussions; the once-clear boundary between engineering progress and ecological impact is becoming increasingly blurred. If you want to complete your Thermodynamic assignment, we must consider how thermodynamic principles can be harnessed to develop sustainable engineering solutions that mitigate environmental harm while still advancing technological innovation.
The significance of thermodynamic processes in this evolving context extends beyond mere mechanical efficiency—it is intrinsically tied to the urgent need for sustainable engineering practices. Engineers are confronted with the challenge of reconciling the demand for enhanced performance with the imperative to reduce environmental footprints. The heightened awareness of finite resources and the adverse effects of greenhouse gas emissions has sparked a collective realization within the engineering community. It is no longer sufficient for mechanical systems to be technically proficient; they must also be ecologically responsible.
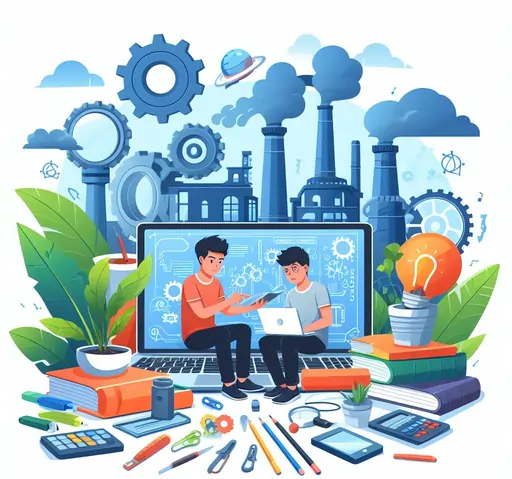
A pivotal aspect of this transformation involves a meticulous analysis of the environmental implications associated with thermodynamic processes. As energy demand continues its upward trajectory on a global scale, engineers are tasked not only with optimizing the performance of mechanical systems but also with minimizing their ecological impact. The environmental concerns span a spectrum, encompassing issues such as resource depletion, air and water pollution, and the overarching specter of climate change. The once-dismissed byproducts of mechanical ingenuity are now at the forefront of engineering considerations, demanding innovative solutions that prioritize the health of the planet.
The quest for sustainable engineering solutions propels engineers into uncharted territories where the traditional trade-offs between efficiency and environmental impact are being reevaluated. It involves a paradigmatic shift towards cleaner energy sources, the adoption of circular economy principles, and the integration of cutting-edge technologies that minimize waste and emissions. From the inception of a design to its operational phase and eventual decommissioning, sustainability is becoming a guiding principle throughout the life cycle of mechanical systems.
This paradigm shift is reflected in the growing prominence of renewable energy sources within thermodynamic processes. The incorporation of solar, wind, and other clean energy technologies into the mechanical engineering landscape symbolizes a departure from the status quo. Engineers are now tasked with not only optimizing the efficiency of systems but also with aligning them with the imperatives of a sustainable future.
In conclusion, the significance of thermodynamic processes in mechanical engineering is undergoing a profound reevaluation. The once-insular realm of mechanical efficiency is expanding to encompass environmental sustainability as a non-negotiable criterion for engineering success. As concerns for our planet's well-being intensify, engineers find themselves at the vanguard of a transformative movement, where the optimization of thermodynamic processes converges with the imperative to forge a more sustainable and harmonious relationship between technology and the environment.
Overview of Thermodynamic Processes:
Fundamental thermodynamic processes form the cornerstone of mechanical engineering, governing the interactions of energy within systems and elucidating the underlying principles that enable the design and operation of a myriad of mechanical devices. These processes, rooted in the laws of thermodynamics, include isothermal, adiabatic, isobaric, and isochoric processes, each delineating distinct transformations of energy within a system.
The isothermal process occurs at constant temperature, whereby a system exchanges heat with its surroundings to maintain a constant temperature. This process is exemplified in applications such as refrigeration systems, where the compression and expansion of refrigerants take place while keeping the temperature constant, ensuring the efficient transfer of heat.
Adiabatic processes, in contrast, transpire without heat exchange with the surroundings. Here, the energy transfer occurs solely through work done on or by the system. Adiabatic compression and expansion are integral to the functionality of internal combustion engines, such as those in automobiles, where the rapid compression and expansion of gases drive the pistons and generate mechanical work.
Isobaric processes involve constant pressure, allowing systems to exchange heat with their surroundings while maintaining a consistent pressure level. This type of process finds application in various engineering scenarios, such as the combustion processes in gas turbines. The continuous flow of gases at constant pressure enables sustained power generation, making isobaric processes indispensable in energy conversion systems.
Isochoric processes, also known as constant volume processes, occur when the volume of a system remains unchanged. While less common in practice, isochoric processes are crucial in specific applications, such as the ignition phase of internal combustion engines. The confined space within the engine cylinder experiences combustion at a constant volume, leading to the rapid generation of high pressures and temperatures.
Widespread use of these fundamental thermodynamic processes permeates various mechanical engineering applications, shaping the design and functionality of systems that power our modern world. Internal combustion engines, central to automotive propulsion, rely on adiabatic compression and expansion processes. Gas turbines in power plants and aircraft engines exploit isobaric processes to efficiently generate power. Refrigeration and air conditioning systems employ isothermal processes to control temperature, ensuring comfort and preserving perishable goods. Even fundamental heat exchangers in everyday appliances and industrial plants leverage these processes to facilitate efficient heat transfer.
In essence, the comprehension and application of fundamental thermodynamic processes empower mechanical engineers to harness and manipulate energy in diverse ways, driving innovation across a spectrum of applications. From enhancing the efficiency of power generation to optimizing the performance of everyday appliances, the mastery of these processes is indispensable for advancing the field of mechanical engineering and addressing the evolving challenges of sustainability in the 21st century.
Environmental Impact Assessment:
The environmental impact of traditional thermodynamic processes constitutes a critical facet of the discourse on sustainable engineering, as these processes have historically been associated with significant ecological consequences. One primary concern revolves around energy consumption, where traditional systems often exhibit inefficiencies leading to excessive energy usage. In conventional power generation, for example, fossil fuel-based thermodynamic processes suffer from inherent inefficiencies in converting fuel energy into electricity. The result is heightened demand for non-renewable resources, exacerbating the depletion of finite energy reserves and contributing to a carbon-intensive energy landscape.
Emissions constitute another pivotal factor in the environmental impact of traditional thermodynamic processes. The combustion of fossil fuels, a prevalent practice in power generation and transportation, releases a plethora of pollutants, including carbon dioxide (CO2), sulfur dioxide (SO2), nitrogen oxides (NOx), and particulate matter. These emissions not only degrade air quality but also contribute to global warming and climate change. The accumulation of greenhouse gases in the atmosphere, particularly CO2, intensifies the greenhouse effect, trapping heat and leading to adverse effects such as rising temperatures, extreme weather events, and sea-level rise.
Moreover, traditional thermodynamic processes often entail resource depletion, further amplifying their environmental footprint. The extraction, processing, and utilization of fossil fuels and other non-renewable resources contribute to habitat destruction, ecosystem disruption, and water pollution. Additionally, the mining of minerals and metals for components in thermodynamic systems can result in soil degradation and biodiversity loss, further compromising the delicate balance of ecosystems.
The environmental impact is not confined solely to the operational phase of traditional thermodynamic processes; the entire life cycle must be scrutinized. The manufacturing, construction, and decommissioning of thermodynamic systems require significant energy inputs, raw materials, and generate waste. The disposal of obsolete or inefficient equipment poses challenges in waste management and often contributes to electronic waste (e-waste) concerns, where hazardous materials can leach into the environment if not properly handled.
As the world increasingly recognizes the urgency of addressing climate change and minimizing environmental degradation, there is a growing impetus to transition away from traditional thermodynamic processes with their substantial environmental footprints. The imperative is to embrace cleaner and more sustainable alternatives, such as renewable energy sources and advanced technologies that optimize energy efficiency. In doing so, the engineering community seeks not only to mitigate the adverse environmental impact of traditional thermodynamic processes but also to pave the way for a more sustainable and ecologically harmonious future.
Case Studies:
Case studies serve as illuminating windows into the tangible environmental consequences of specific thermodynamic processes, offering insights into the sustainability challenges and opportunities inherent in various engineering choices. One notable case study involves conventional coal-fired power plants, a prevalent source of electricity globally. The combustion of coal releases substantial quantities of carbon dioxide, sulfur dioxide, and nitrogen oxides, contributing significantly to air pollution and climate change. The environmental impact extends to resource extraction, where coal mining leads to habitat destruction, soil erosion, and water pollution. In contrast, a case study on a solar thermal power plant showcases a more sustainable alternative. Solar thermal technology harnesses the sun's energy to generate electricity without emitting greenhouse gases during operation. Although the production and disposal of solar panels pose environmental challenges, the overall life cycle impact is considerably lower compared to traditional coal-fired plants.
Agricultural processes also exemplify the environmental ramifications of specific thermodynamic choices. The widespread use of traditional irrigation systems, often powered by fossil fuel-driven pumps, poses challenges related to energy consumption and emissions. The extraction of groundwater for irrigation can lead to resource depletion and land subsidence. In contrast, a case study on the implementation of sustainable irrigation practices utilizing solar-powered pumps illustrates a more environmentally conscious approach. By harnessing renewable energy, these systems reduce dependence on fossil fuels, minimize emissions, and mitigate the environmental impact associated with conventional irrigation methods.
Comparing the sustainability of different thermodynamic processes and technologies reveals the imperative for a paradigm shift towards greener alternatives. In the realm of transportation, a comparison between internal combustion engines and electric vehicles highlights the stark differences in environmental impact. Internal combustion engines rely on fossil fuels, leading to emissions of pollutants and greenhouse gases. On the other hand, electric vehicles powered by renewable energy sources offer a cleaner and more sustainable option, reducing air pollution and dependence on finite resources.
In the context of industrial heating processes, the choice between traditional fossil fuel-based systems and innovative biomass heating systems underscores the importance of sustainable alternatives. Biomass systems utilize organic materials, often waste products, to generate heat, offering a renewable and carbon-neutral energy source. This stands in contrast to traditional heating processes that rely on fossil fuels, contributing to emissions and resource depletion.
Ultimately, the comparison of case studies underscores the pivotal role of engineering choices in shaping the environmental impact of thermodynamic processes. Sustainable alternatives, characterized by reduced emissions, minimized resource depletion, and reliance on renewable energy sources, emerge as crucial pathways towards a more environmentally conscious future. As industries and societies continue to grapple with the challenges of sustainability, these case studies provide valuable insights for informed decision-making and the pursuit of greener engineering practices.
Sustainable Engineering Solutions:
Sustainable engineering practices stand as a paramount solution to address and mitigate the environmental impact of traditional thermodynamic processes, ushering in an era where technological advancements align with ecological stewardship. At the core of sustainable engineering is the commitment to balance the pursuit of efficiency and innovation with the imperative to minimize adverse environmental effects. One key aspect of this paradigm shift involves reimagining thermodynamic processes through innovations that prioritize environmental sustainability. Advanced technologies have emerged to optimize energy efficiency and reduce the ecological footprint of mechanical systems. For instance, Combined Heat and Power (CHP) systems, also known as cogeneration, represent a notable innovation. These systems simultaneously generate electricity and useful heat from the same energy source, enhancing overall efficiency compared to conventional separate power and heat production. Additionally, advancements in waste heat recovery technologies have gained prominence, allowing industries to capture and repurpose excess heat from various processes, further improving energy efficiency and reducing waste.
In the pursuit of sustainable thermodynamic processes, the integration of renewable energy sources has become a hallmark of innovation. Solar thermal systems, for example, harness sunlight to produce heat, offering a clean and abundant energy source for various applications. Innovations in solar technologies, such as concentrating solar power (CSP) and advanced photovoltaics, continue to enhance the efficiency and cost-effectiveness of solar energy utilization in thermodynamic processes. Similarly, advancements in wind turbine technology contribute to sustainable power generation, harnessing the kinetic energy of the wind to produce electricity with minimal environmental impact.
In the realm of sustainable transportation, electric propulsion systems represent a transformative innovation. Electric vehicles (EVs), powered by batteries or fuel cells, are gradually replacing traditional internal combustion engines, reducing reliance on fossil fuels and mitigating emissions. The development of high-capacity batteries and improvements in charging infrastructure are key advancements driving the widespread adoption of electric vehicles, transforming the automotive industry and contributing to a more sustainable future.
Furthermore, the exploration of innovative materials plays a pivotal role in sustainable thermodynamic processes. The use of eco-friendly and recyclable materials in the construction of mechanical systems reduces environmental impact across their life cycle. Advances in materials science contribute to the development of components that are durable, energy-efficient, and environmentally responsible, fostering a holistic approach to sustainable engineering.
In conclusion, sustainable engineering practices offer a transformative solution to the environmental challenges posed by traditional thermodynamic processes. The integration of innovative technologies, the harnessing of renewable energy sources, and the exploration of eco-friendly materials collectively propel the field of mechanical engineering towards a future where efficiency and environmental responsibility coexist. As these sustainable advancements continue to evolve, they underscore the potential for engineering to be a driving force in mitigating environmental impact and fostering a more sustainable and resilient global infrastructure.
Renewable Energy Integration:
The integration of renewable energy sources into thermodynamic processes marks a pivotal stride towards a sustainable and eco-friendly future in mechanical engineering. Harnessing the power of renewable resources such as solar, wind, hydro, and geothermal energy involves innovative approaches to ensure their seamless incorporation into traditional thermodynamic systems. Solar energy, for instance, can be integrated into thermodynamic processes through technologies like solar thermal systems and photovoltaics. In solar thermal systems, concentrated sunlight is used to produce high-temperature heat, which can be employed in various industrial processes or for power generation. Photovoltaic cells directly convert sunlight into electricity, providing a clean and sustainable power source for mechanical systems. Wind energy, another renewable powerhouse, can be harnessed through wind turbines to generate electricity that complements or substitutes for conventional power sources in thermodynamic processes.
One of the primary benefits of utilizing clean energy in mechanical engineering applications lies in the drastic reduction of greenhouse gas emissions and pollutants. Unlike traditional thermodynamic processes reliant on fossil fuels, renewable energy sources produce little to no emissions during operation, mitigating air pollution and curbing the contribution to climate change. The transition to clean energy not only addresses environmental concerns but also aligns with global efforts to meet emission reduction targets and combat the adverse impacts of climate change.
Moreover, the sustainability of renewable energy sources ensures a reliable and long-term energy supply, reducing dependence on finite and environmentally damaging resources. In contrast to fossil fuels that deplete over time and contribute to geopolitical tensions, renewable resources are abundant, widely distributed, and can be harnessed locally. This decentralization of energy production enhances energy security and resilience in the face of global challenges.
The economic advantages of incorporating renewable energy into thermodynamic processes further underscore the merits of this transition. While the initial investment in renewable infrastructure may be higher, the long-term operational costs tend to be lower, particularly as advancements in technology continue to improve efficiency and reduce the cost of renewable energy systems. Additionally, the renewable energy sector fosters job creation and stimulates economic growth, contributing to a sustainable and thriving industry.
Furthermore, the utilization of clean energy enhances the social and environmental responsibility of mechanical engineering practices. Industries adopting renewable energy not only contribute to a healthier planet but also position themselves as leaders in corporate social responsibility, responding to the increasing demands of environmentally conscious consumers and stakeholders.
In conclusion, integrating renewable energy sources into thermodynamic processes heralds a transformative era in mechanical engineering, promising a cleaner, more sustainable, and economically viable future. Beyond the immediate environmental benefits, this transition catalyzes innovation, fosters energy security, and aligns with the global imperative to create a resilient and sustainable energy landscape for generations to come.
Life Cycle Analysis:
Life Cycle Analysis (LCA) plays a pivotal role in evaluating the overall environmental impact of products, processes, or systems throughout their entire life cycle — from raw material extraction and manufacturing to use, and eventual disposal or recycling. This holistic approach is crucial in providing a comprehensive understanding of the environmental consequences associated with a particular engineering decision. LCA takes into account various factors, including resource consumption, energy use, emissions, and waste generation, allowing engineers to quantify and compare the environmental burdens associated with different options.
The importance of life cycle analysis lies in its ability to uncover hidden environmental impacts that may not be immediately apparent when focusing solely on one phase of a product or process. By considering the entire life cycle, engineers gain insights into the cumulative effects and trade-offs that may exist between different stages. For instance, a product with a low environmental impact during its use phase might have significant impacts during its manufacturing or disposal phases. LCA thus provides a holistic perspective, preventing the shifting of environmental burdens from one stage to another, known as "problem shifting."
Engineers can use life cycle assessment tools to make informed decisions by following a systematic and standardized methodology. The process typically involves four key stages: goal and scope definition, inventory analysis, impact assessment, and interpretation. In the goal and scope definition stage, engineers establish the objectives of the assessment and define the boundaries of the system under study. The inventory analysis involves collecting data on resource inputs and outputs at each stage of the life cycle. This data forms the basis for assessing the environmental impacts in the next stage, where impact categories such as greenhouse gas emissions, water consumption, and toxicity are evaluated. The final stage involves interpreting the results in the context of the study's goals and scope.
Software tools specifically designed for life cycle assessment, such as SimaPro, GaBi, or openLCA, facilitate the application of LCA methodologies. These tools streamline data collection, analysis, and interpretation, making the process more accessible to engineers. Additionally, databases integrated into these tools provide a wealth of information on the environmental profiles of various materials and processes, enhancing the accuracy and reliability of the assessments.
By employing life cycle assessment tools, engineers can identify opportunities for improvement and optimization at different stages of a product or process. This might involve selecting more sustainable materials, improving energy efficiency during manufacturing, or implementing recycling programs at the end of a product's life. LCA empowers engineers to make informed decisions that prioritize environmental sustainability, aligning with the broader goals of responsible and conscientious engineering practices. As industries and consumers increasingly demand eco-friendly solutions, life cycle analysis emerges as an indispensable tool for engineers navigating the complexities of designing and implementing environmentally conscious technologies.
Regulatory Frameworks and Standards:
Existing regulations and standards related to the environmental impact of engineering processes are crucial components of the global effort to promote sustainability and mitigate environmental degradation. Various international, national, and industry-specific standards address key aspects of environmental impact, ranging from emissions and waste management to resource conservation and energy efficiency.
One prominent set of regulations is the ISO 14000 family of standards, with ISO 14001 being the most widely recognized. ISO 14001 outlines the criteria for an environmental management system, providing a framework for organizations to effectively manage their environmental responsibilities. Compliance with ISO 14001 is voluntary but signals a commitment to proactively addressing environmental impact and continually improving environmental performance.
At the national level, countries often have their own environmental regulations that engineering processes must adhere to. For instance, the U.S. Environmental Protection Agency (EPA) sets standards for air and water quality, waste disposal, and hazardous substance management. Similarly, the European Union's Eco-Management and Audit Scheme (EMAS) establishes requirements for organizations to assess, manage, and improve their environmental performance.
In the realm of specific industries, such as manufacturing or construction, standards like the LEED (Leadership in Energy and Environmental Design) certification for green building practices or the Responsible Care program in the chemical industry provide guidelines for minimizing environmental impact.
The role of compliance in promoting sustainable practices is multifaceted. Firstly, regulatory frameworks establish baseline expectations, setting minimum standards that companies must meet to operate legally. This serves as a deterrent to environmentally harmful practices and creates a level playing field, ensuring that all entities within a sector adhere to a common set of environmental responsibilities.
Compliance also acts as a catalyst for continuous improvement. Organizations striving to meet and exceed regulatory requirements often implement measures that go beyond mere compliance. This proactive approach fosters innovation, encouraging the development and adoption of cleaner technologies, processes, and materials. It positions compliance as a starting point rather than a finish line, emphasizing the dynamic nature of sustainable practices.
Moreover, compliance enhances transparency and accountability. Companies are required to monitor, report, and verify their environmental performance, making this information accessible to regulators, stakeholders, and the public. This transparency fosters trust and facilitates informed decision-making by consumers, investors, and regulatory authorities.
While compliance is essential, it is increasingly complemented by voluntary sustainability initiatives. Many organizations choose to adopt certifications and labels that go beyond legal requirements, signaling their commitment to environmental stewardship. These initiatives, such as the Forest Stewardship Council (FSC) certification for responsible forestry practices or Cradle to Cradle certification for environmentally safe products, provide additional frameworks for companies to demonstrate their dedication to sustainability.
In conclusion, existing regulations and standards form the backbone of efforts to mitigate the environmental impact of engineering processes. Compliance plays a pivotal role in shaping sustainable practices by establishing benchmarks, encouraging continuous improvement, fostering transparency, and providing a foundation for voluntary initiatives that push the boundaries of environmental responsibility in engineering.
Conclusion:
The blog delves into the critical theme of "Environmental Impact of Thermodynamic Processes: Analysis for Sustainable Engineering Solutions." It underscores the foundational role of thermodynamic processes in mechanical engineering, acknowledging their significance in powering modern systems while highlighting the escalating concern for environmental sustainability. The analysis spans key components, such as energy consumption, emissions, and resource depletion, elucidating the environmental consequences of traditional thermodynamic practices. Sustainable engineering practices emerge as a paramount solution, emphasizing the need for a paradigm shift. Life Cycle Analysis (LCA) is spotlighted as a pivotal tool for evaluating the overall environmental impact, offering a comprehensive understanding of the consequences throughout a product's life cycle. The integration of renewable energy sources into thermodynamic processes is championed as a transformative solution, with solar, wind, and other clean energy technologies leading the charge. The importance of regulations and standards is emphasized, showcasing how compliance acts as a catalyst for sustainable practices, setting baseline expectations, and fostering continuous improvement.
In the context of this discourse, the role of mechanical engineers is paramount in contributing to sustainable practices. Engineers are positioned as key agents of change, capable of driving innovations and advancements that align with environmental responsibility. The narrative underscores the engineer's capacity to influence the entire life cycle of products and systems, from design to disposal. Integration of renewable energy sources, adoption of cleaner technologies, and adherence to sustainable engineering principles become avenues through which mechanical engineers can steer their projects towards eco-friendly outcomes. The responsibility extends beyond technical acumen to a holistic understanding of the broader environmental impact, necessitating a commitment to sustainable decision-making and a proactive stance in the face of evolving challenges.
As a call to action, the blog encourages readers, especially those involved in engineering projects, to explore and implement eco-friendly solutions. It emphasizes the collective responsibility to contribute to a sustainable future, urging engineers to leverage their skills and creativity for environmentally conscious endeavors. The exploration of renewable energy sources, adoption of life cycle assessment methodologies, and adherence to recognized standards are positioned as accessible and impactful steps. The overarching message is one of empowerment — the acknowledgment that every engineering project offers an opportunity to make choices that resonate with sustainability. By embracing these choices, engineers can play a pivotal role in mitigating environmental impact and steering the trajectory of mechanical engineering towards a cleaner, more sustainable, and resilient future.