Using Thermodynamics in Material Science: Understanding Properties and Changes!
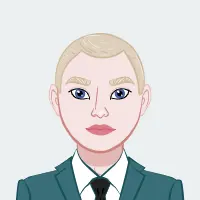
Thermodynamics serves as an indispensable cornerstone in the realm of material science, wielding its influence over the understanding of properties and changes inherent in diverse materials. At its core, thermodynamics is a discipline that investigates the fundamental principles governing energy and matter interactions. In the context of material science, these interactions are pivotal to comprehending the behavior, characteristics, and alterations that materials undergo. The profound importance of thermodynamics lies in its ability to provide a systematic framework for analyzing and predicting the intricate relationships between energy, heat, and the structural configurations of materials. If you need assistance with your thermodynamics assignment, feel free to ask for clarification or guidance on specific concepts.
The first law of thermodynamics, often referred to as the law of conservation of energy, asserts that energy cannot be created nor destroyed but can only change forms. This principle is particularly pertinent to material science, where understanding the conservation and transformation of energy is paramount. The second law, delving into the concept of entropy, elucidates the natural tendency of systems to move towards states of greater disorder. In the context of material science, this law sheds light on the spontaneous evolution of materials, guiding researchers in comprehending the inherent changes and transitions.
Furthermore, thermodynamics provides a crucial lens through which to examine the properties of materials. Whether assessing the stability of a given material under specific conditions or discerning the optimal parameters for a manufacturing process, thermodynamics offers a rigorous methodology. The equilibrium state of a material, dictated by thermodynamic principles, elucidates its intrinsic properties such as density, elasticity, and thermal conductivity. By applying these principles, scientists and engineers gain a nuanced understanding of how materials respond to external stimuli, be it changes in temperature, pressure, or composition.
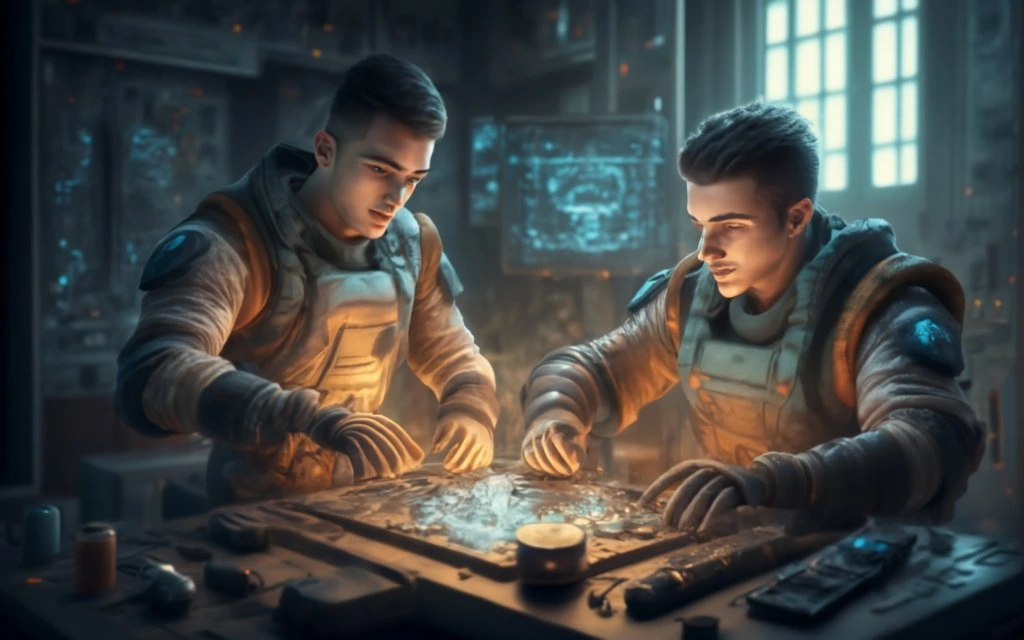
In the dynamic field of material science, where innovations often hinge on manipulating and tailoring the properties of materials to meet specific needs, thermodynamics becomes an invaluable tool. The understanding of phase transitions, for instance, is deeply rooted in thermodynamic principles. Whether transitioning from solid to liquid or experiencing more intricate transformations, materials' behavior can be predicted and controlled through the application of thermodynamics. This predictive power extends to diverse material changes, including alloying, heat treatment, and crystallization, offering engineers and researchers the ability to optimize material performance.
The role of thermodynamics in material science extends beyond static analyses; it actively contributes to the development of groundbreaking technologies. From the synthesis of new materials with tailored properties to the optimization of existing ones for enhanced performance, thermodynamics guides researchers in the quest for materials that push the boundaries of what is possible. The systematic application of thermodynamic principles has, for example, led to the discovery of novel materials with superior strength, conductivity, and resilience, revolutionizing industries ranging from electronics to aerospace.
In conclusion, thermodynamics stands as an indispensable foundation in the field of material science, providing a systematic approach to unraveling the complexities of energy and matter interactions. Its principles not only enable the understanding of materials' properties but also empower scientists and engineers to predict and manipulate material changes. As advancements in material science continue to shape technological landscapes, thermodynamics remains an enduring guide, steering the course towards innovative materials that redefine the boundaries of possibility.
Basics of Thermodynamics:
Thermodynamics, a cornerstone of classical physics, encompasses a set of fundamental principles governing the behavior of energy and matter in physical systems. At its core are the four laws of thermodynamics, which form the theoretical framework underpinning the science. The first law, often known as the law of energy conservation, posits that energy within an isolated system remains constant—energy may change forms but is never created nor destroyed. This principle provides the foundation for understanding energy transfer and transformations within various processes.
The second law introduces the concept of entropy, a measure of the system's disorder or randomness. It states that natural processes tend to increase the overall entropy of a closed system, reflecting a spontaneous move toward states of greater disorder. Entropy's significance lies in its role as a guiding principle in determining the directionality of physical processes. For instance, in material science, entropy sheds light on the natural progression of materials towards equilibrium states and the inherent tendency for systems to evolve from order to disorder.
The third law of thermodynamics establishes the unattainability of absolute zero, the lowest possible temperature. As a system approaches absolute zero, entropy approaches a minimum value, and the molecular motion ceases. This law underscores the impossibility of reaching absolute zero through any finite process, providing insights into the behavior of matter at extremely low temperatures.
Energy transfer, a central theme in thermodynamics, manifests through various mechanisms, such as heat transfer and work done. Heat transfer occurs when energy moves between systems due to a temperature difference, while work done involves the application of force to move an object. Both processes are integral to understanding and quantifying energy exchanges in different scenarios.
Thermodynamics further classifies systems into open, closed, and isolated systems, each with distinct characteristics regarding the exchange of matter and energy with their surroundings. This classification is pivotal for analyzing real-world processes, providing a framework for understanding how energy and matter flow in and out of a system.
In conclusion, the fundamental principles of thermodynamics form a comprehensive and structured approach to studying energy and matter interactions. The laws of thermodynamics, encompassing energy conservation, entropy, and the unattainability of absolute zero, serve as guiding principles. These laws, coupled with an understanding of energy transfer mechanisms and system classifications, provide a robust foundation for exploring diverse phenomena in physics, chemistry, and engineering, making thermodynamics an essential discipline with broad applications in scientific and technological domains.
Application of Thermodynamics in Material Science:
Thermodynamics, a key discipline in material science, is applied with precision to analyze and predict the behavior of materials, offering insights into their properties and transformations under varying conditions. The relationship between thermodynamic principles and material properties is deeply ingrained, providing a systematic framework for understanding and manipulating the intricate interactions within diverse materials.
One pivotal application of thermodynamics in material science lies in the prediction of phase transitions. By scrutinizing the thermodynamic conditions that dictate transitions between solid, liquid, and gaseous states, researchers can foresee how materials respond to changes in temperature and pressure. This insight is indispensable for designing and optimizing materials for specific applications. For instance, in metallurgy, understanding the thermodynamics of phase transformations is fundamental to tailoring the mechanical properties of alloys and achieving desired microstructures through heat treatment processes.
Thermodynamics is also instrumental in elucidating the equilibrium state of materials. By analyzing the relationships between temperature, pressure, and material properties, researchers can identify the conditions under which a material is stable and exhibits optimal properties. This knowledge is harnessed in fields such as materials engineering, where the thermodynamic understanding of equilibrium states guides the selection of materials for specific applications, ensuring durability, efficiency, and functionality.
The relationship between thermodynamics and material properties extends to the study of thermodynamic potentials, such as Gibbs free energy. These potentials provide critical information about the spontaneity of reactions and the stability of materials. Engineers and scientists leverage this knowledge to predict how materials will behave under different environmental conditions, facilitating the design of materials that are resistant to corrosion, degradation, or fatigue.
Furthermore, thermodynamics aids in the understanding of material transport phenomena, such as diffusion and mass transfer. By considering the thermodynamic driving forces behind these processes, researchers can anticipate how materials will interact and evolve over time, crucial for applications ranging from material degradation to the controlled release of substances in pharmaceuticals and nanotechnology.
In essence, the application of thermodynamics to analyze and predict material behavior is rooted in its capacity to unravel the complex interplay between energy, entropy, and material properties. The principles of thermodynamics offer a predictive lens through which researchers and engineers can navigate the vast landscape of material science, guiding the development of new materials with tailored properties and functionality. As the synergy between thermodynamics and material science continues to evolve, it propels advancements in diverse industries, paving the way for innovative materials that meet the demands of contemporary technology and engineering challenges.
Understanding Material Changes:
Thermodynamics serves as a powerful analytical tool for understanding material changes, with specific applications evident in phenomena such as phase transitions, heat treatment, and other relevant processes. Phase transitions, a key focus in material science, exemplify the profound impact of thermodynamics on material behavior. By scrutinizing the thermodynamic conditions governing transitions between solid, liquid, and gaseous states, researchers can unravel the intricacies of material transformations. For instance, the understanding of phase diagrams, which depict the equilibrium phases of a material at varying temperature and pressure, enables engineers to predict and control transitions. In the realm of metallurgy, the application of thermodynamics is particularly pronounced, guiding the design of alloys with tailored mechanical properties through careful consideration of phase equilibria.
Heat treatment, another critical facet of material science, underscores the transformative role of thermodynamics in altering material properties. By subjecting materials to controlled heating and cooling processes, engineers harness thermodynamic principles to manipulate the microstructure of materials, thereby influencing mechanical and thermal properties. Heat treatment encompasses processes like annealing, quenching, and tempering, each guided by specific thermodynamic considerations. For instance, during annealing, materials are heated to promote recovery or recrystallization, and thermodynamics guides the optimal conditions for achieving desired microstructural changes, influencing properties such as hardness and ductility.
Moreover, thermodynamics plays a pivotal role in elucidating other relevant processes that drive material changes. The concept of Gibbs free energy, a thermodynamic potential, is often employed to determine the spontaneity of reactions and the stability of materials. Engineers leverage this understanding to predict whether a material will undergo corrosion, degradation, or other transformative processes under particular environmental conditions. Thermodynamics also guides the study of diffusion, where the movement of atoms or molecules within a material is driven by thermodynamic gradients. This is crucial in understanding processes like material aging, where diffusion phenomena influence structural changes over time.
In conclusion, the application of thermodynamics in understanding material changes is exemplified through its impact on phase transitions, heat treatment, and various relevant processes. The precision offered by thermodynamics allows researchers and engineers to not only predict these changes but also strategically manipulate material properties for specific applications. As advancements in material science continue to unfold, the synergy between thermodynamics and the understanding of material changes remains a cornerstone, paving the way for innovative materials with enhanced functionality and performance across diverse industries.
Challenges and Future Trends:
Despite its pivotal role in material science, the application of thermodynamics is not without challenges. One significant challenge lies in the complexity of real-world systems, which often deviate from idealized conditions assumed in thermodynamic models. Achieving accurate predictions for intricate materials and processes is hindered by the presence of impurities, defects, and non-equilibrium conditions, requiring researchers to develop advanced models that account for these complexities. Additionally, experimental limitations in measuring thermodynamic properties, especially at extreme conditions, pose challenges in validating and refining theoretical predictions. The integration of quantum mechanics into thermodynamic models is another challenge, as materials at the atomic and molecular levels often exhibit quantum behaviors that classical thermodynamics struggles to capture.
Looking ahead, emerging trends and future developments in the integration of thermodynamics with material science hold promising prospects. The advent of computational tools and simulations, coupled with advances in machine learning and artificial intelligence, enables researchers to model and predict material behavior with unprecedented accuracy. This computational prowess facilitates the exploration of complex thermodynamic interactions in materials, overcoming some of the challenges associated with real-world complexities. The integration of multiscale modeling, incorporating both macroscopic and microscopic perspectives, is gaining traction, allowing for a more comprehensive understanding of thermodynamic processes within materials.
Furthermore, there is a growing emphasis on incorporating thermodynamics into the design of materials with specific functionalities. Tailoring materials for optimal performance requires a deep understanding of the thermodynamic principles governing their behavior. The integration of thermodynamics into the development of smart materials, responsive to external stimuli, opens avenues for innovative applications in fields such as electronics, energy storage, and medicine. Sustainable materials design is also a key focus, with thermodynamics playing a central role in optimizing processes to minimize energy consumption and environmental impact.
In the quest for materials with unprecedented properties, the exploration of non-equilibrium thermodynamics is becoming increasingly important. Understanding and manipulating materials far from thermodynamic equilibrium conditions offers new possibilities for creating novel materials and achieving desirable properties not achievable in equilibrium states. This shift towards non-equilibrium thermodynamics aligns with the dynamic nature of modern materials science and opens up avenues for breakthroughs in areas such as rapid solidification, high-speed processing, and innovative manufacturing techniques.
In conclusion, while challenges persist in applying thermodynamics to material science, ongoing advancements in computational capabilities and a shift towards integrating thermodynamics into materials design are shaping the future landscape. The incorporation of multiscale modeling, the exploration of non-equilibrium thermodynamics, and the convergence of thermodynamics with emerging technologies are poised to revolutionize the field, paving the way for the development of materials with unprecedented properties and functionalities.
Conclusion:
In this blog post, we delved into the profound connection between thermodynamics and material science, emphasizing how thermodynamics serves as an invaluable tool for analyzing and predicting material behavior. The fundamental principles of thermodynamics, including the laws of energy conservation and entropy, provide a structured framework for understanding the intricate interactions within diverse materials. By applying these principles, researchers can gain insights into the properties, changes, and transformations materials undergo. Specific applications of thermodynamics, such as predicting phase transitions, optimizing material properties through heat treatment, and elucidating relevant processes like diffusion, were explored. We highlighted the challenges associated with applying thermodynamics in real-world scenarios, including the complexities of non-ideal conditions and the limitations in experimental measurements. Despite these challenges, the blog underscored the ongoing advancements in computational tools, machine learning, and multiscale modeling that are enhancing our ability to model and predict material behavior more accurately.
Looking to the future, the integration of thermodynamics with material science is poised for exciting developments. Computational capabilities and the use of artificial intelligence are enabling researchers to overcome challenges associated with complex systems, paving the way for more accurate predictions and a deeper understanding of materials. The emphasis on incorporating thermodynamics into the design of materials for specific functionalities, such as in smart materials and sustainable design, suggests a paradigm shift towards more intentional and purposeful material development. The exploration of non-equilibrium thermodynamics is identified as a key area of interest, offering new possibilities for creating novel materials with unprecedented properties.
To readers, we encourage further exploration of the intricate connection between thermodynamics and material science for a more profound understanding of these fundamental principles. The synergy between these disciplines not only shapes our comprehension of material behavior but also drives innovations in various industries. Whether you are a student, researcher, or professional in the field, delving deeper into the application of thermodynamics in material science can unlock new perspectives and insights. As technology continues to advance and new challenges emerge, the integration of thermodynamics with material science stands at the forefront, guiding the development of materials that are not only resilient and efficient but also tailored for specific applications in our rapidly evolving world.